International Decade of Sciences for Sustainable Development (IDSSD)
The Earth-Humanity Coalition (EHC)
World Academy of Art and Science (WAAS)
New Carbon Economy Consortium (NCEC)
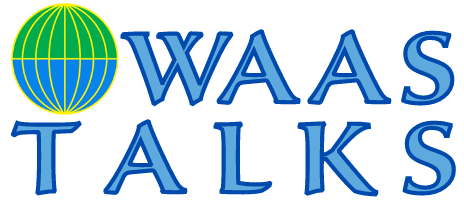
Online on February 20, 2025 from 6:00 pm to 7:35 pm CET
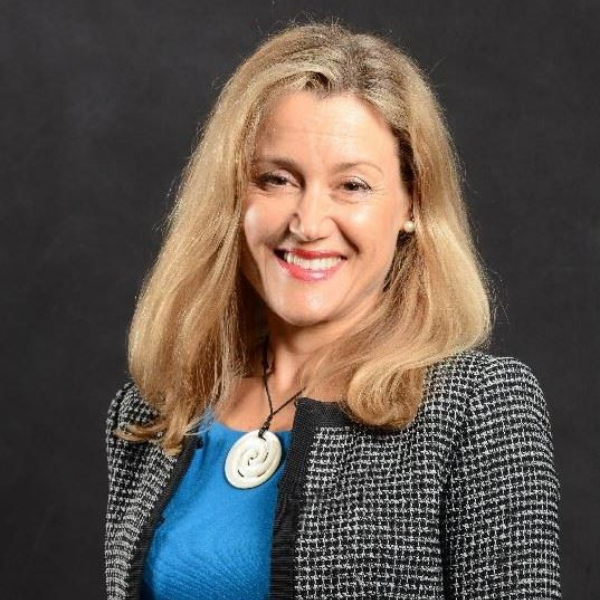
Amanda Ellis
Senior Director for Strategic Partnerships and Networks, Julie Ann Wrigley Global Futures Laboratory, Executive Director for Asia and Pacific, Global Institute of Sustainability and Innovation, Professor of Practice, Thunderbird School of Global Management – Arizona State University, Tempe and Phoenix, USA; Trustee, World Academy of Art and Science (WAAS); Amanda.Natalie.Ellis@asu.edu
Opening and Moderation
In August 2023, the UN General Assembly proclaimed the International Decade of Sciences for Sustainable Development (IDSSD), from 2024 to 2031. The task to lead the preparation and implementation of the activities within IDSSD was given to UNESCO. On April 16, 2024, The Earth-Humanity Coalition (EHC) was founded – as an association of global, regional, and national scientific organizations with the task to prepare and implement, in close cooperation with UNESCO, various initiatives within the overall program of IDSSD. The World Academy of Art and Science (WAAS) was among the founding Members of EHC. It had initiated a program of sciences for sustainable development, which became an EHC specific initiative – the EHC-WAAS Program of Sciences for Sustainable Development.
The WAAS Talks on Science for Humans Security: New Carbon Economy is the sixth webinar within the Program. It had been initiated by Amanda Ellis, Co-Chair of the New Carbon Economy Consortium (NCEC) and Former UN Ambassador and Prime Minister’s Special Envoy for New Zealand; her additional current affiliations are given below her photo. Also, she had chosen the speakers – Klaus S. Lackner, Matthew Green, Frances Wall, and Walter Mérida, whose affiliations are also given below their photos – and moderated the event.
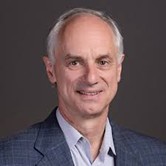
Klaus S. Lackner
Founding Director, Center for Negative Carbon Emissions (CNCE), Professor, School of Sustainable Engineering and the Built Environment – Arizona State University, Tempe, USA; Klaus.Lackner@asu.edu
Talks
New carbon economy
Statements
Carbon dioxide emissions from the energy sector are the primary driver of climate change. While energy efficiency and conservation can help, modern societies depend on energy for development, economic growth, and sustainability. The key challenge is not stopping energy consumption but transitioning to a carbon-neutral system while managing the excess CO2 already in the atmosphere. Current energy infrastructure, based on fossil fuels, is unsustainable. A shift to carbon-neutral energy sources such as solar, nuclear, and fossil fuels with carbon capture and storage is essential. However, even with a rapid transition, past and future emissions must be addressed through large-scale CO2 removal.
CO2 remains in the atmosphere and ocean for millennia, making mitigation alone insufficient. Achieving climate targets, such as limiting warming to 1.5°C, requires negative emissions. At current rates, atmospheric CO2 could exceed 450 ppm within 16 years, necessitating the removal of 100 ppm or more. Scalable negative emissions technologies must be implemented, including afforestation, ocean-based CO2 removal, and direct air capture. Of these, direct air capture is the most flexible and scalable approach, capable of removing CO2 at the required scale while allowing carbon to be permanently stored or reused in synthetic fuels.
Despite its viability, direct air capture faces economic and political hurdles. Critics argue it creates a “moral hazard” by discouraging emissions reductions. However, delaying its adoption only increases future risks. Technological advances have reduced costs, and mass production could lower prices further, making large-scale air capture feasible. A decentralized approach, with millions of small capture units, can achieve global CO2 removal while integrating into existing energy systems.
To succeed, early adoption and voluntary action are crucial. Governments, industries, and consumers must support carbon-neutral fuels and CO2 sequestration incentives. If scaled effectively, air capture can remove over 35 Gt of CO2 annually, ultimately balancing the world’s carbon budget. The transition to a carbon-negative economy requires immediate investment, policy support, and market-driven solutions, ensuring a sustainable future while mitigating the long-term effects of climate change.
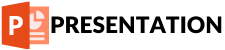
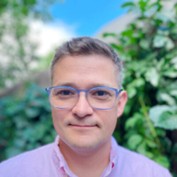
Matthew Green
Director, Center for Negative Carbon Emissions (CNCE), Associate Director, Center for Sustainable Macromolecular Materials and Manufacturing of the Biodesign Institute, Associate Professor, School for Engineering of Matter, Transport and Energy – Arizona State University, Tempe, USA; mdgreen@asu.edu
Direct air capture technology
Statements
A key step toward addressing these challenges lies in innovative materials research. Traditional sorbents often suffer from limited capacity or slow kinetics when targeting low-concentration CO2, highlighting the need for tailor-made polymers. By engineering polymer backbones and functional groups, researchers can design materials with optimized gas transport pathways and enhanced selectivity. High surface area polymers, for instance, provide abundant adsorption sites, improving overall CO2 uptake, while carefully tuned polymer networks can accelerate sorption kinetics. Advances in materials characterization, such as in situ spectroscopic methods, further guide the discovery and rational design of next-generation sorbents and membranes with improved stability, faster response, and lower energy demand.
However, developing advanced materials is only one part of the solution; systems-level design is equally critical for effective CO2 capture and utilization. Membrane-based capture processes would enable continuous capture of CO2, a significant advancement over current batch processes. Scaling up from bench-scale prototypes to commercial facilities requires collaborative efforts in process engineering, reactor design, and life cycle analysis to ensure that deployment is both cost-effective and environmentally sustainable. Incorporating feedback from real-world operation enables robust system architectures that can be readily adapted to diverse operational environments.
The talk highlighted several strategies that united materials research with systems design, including a membrane-based technology to continuously capture CO2, a high surface area polymeric sorbent that controls the kinetics of CO2 sorption, a capture system that can be seamlessly integrated with biological utilization, and more. By combining advanced polymers with holistic process engineering, these emerging technologies represent promising pathways to achieve negative emissions targets and mitigate the most severe impacts of climate change.
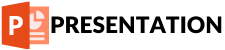
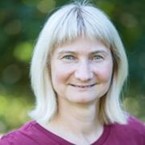
Frances Wall
Professor of Applied Mineralogy, Camborne School of Mines of the University of Exeter, UK; F.Wall@exeter.ac.uk
Critical minerals
Statements
Critical minerals are the ingredients in materials, components and products that make them work. Our modern world puts most of the elements in the Periodic Table to use in some way or other. The most famous critical minerals at the moment are lithium, cobalt, and nickel needed for the lithium-ion batteries in electric vehicles (EVs), energy storage and all our other gadgets, and rare earth elements, required for the permanent magnet motors in EVs and wind turbines. Even though these elements are essential in many applications, we might not need very much of them. The amounts of most critical minerals mined each year are hundreds of times less than copper for example. This means that just a few mines might dominate world supply, and leave the supply chain vulnerable to disruption. China dominates the mining, processing, and manufacturing of many of the critical minerals.
But China does not have a monopoly on deposits of critical minerals and many other counties have natural endowments of minerals that the rest of the world needs. So, while the assessment of critical minerals is a defensive strategy to protect manufacturing industry, countries who have geological deposits now have an opportunity to benefit from them. The ability to benefit is not straightforward given past experience and the so-called ‘resource-curse’ that has accompanied development of natural resources in some countries.
The talk discussed some of the research that was taking place to understand and find new geological deposits, extract more critical minerals as by-products from mines already producing major metals, extract and process in more environmentally-friendly ways, join and create a circular economy, and put the development of resources in a framework of strong sustainable development by using the UN Resource Management System.
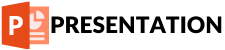
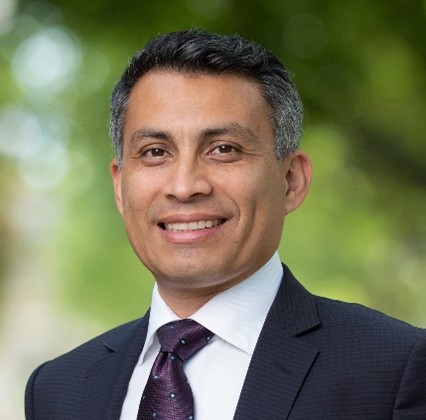
Walter Mérida
Associate Dean, Research & Industry Partnerships, Faculty of Applied Sciences, Leader, Mérida Labs, Professor, Department of Mechanical Engineering – University of British Columbia, Vancouver, Canada; walter.merida@ubc.ca
Hydrogen without CO2: A Living Laboratory using molten-metal methane pyrolysis
Statements
Addressing climate change challenges requires accelerated innovation processes. We have decades – not centuries – to decarbonise the global energy system. In this talk, I will briefly describe the Living Laboratory model that the University of British Columbia has pioneered to address climate change. Out of several on- and off-campus examples, I will focus on a hydrogen project deployed outside the province of British Columbia.
Hydrogen is used in a variety of industrial processes, and it represents a growing global market (US$ 243 billion in 2023). However, most of the current hydrogen production relies on fossil pathways (e.g., steam methane reforming or SMR) with a considerable carbon footprint (SMR produces 7–12 kg CO2/kg H2, representing more than 2% of global emissions). Methane pyrolysis provides a pathway to produce low-cost hydrogen with low- or zero-carbon emissions (2.3–0.7 kg CO2/kg H2 in British Columbia). The solid carbon by-product has several applications (battery electrodes, concrete additives, tyre manufacture, etc.)
Beyond technologies and innovation, university research can amplify academic impact by enabling regional economic development. Demonstrating low- or zero-emission solutions outside the laboratory, can provide an effective platform for dialogue with governments, utilities, indigenous communities, civil society, and organised labour.
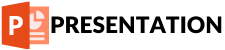